Dynamics and significance of genetic variation in a metapopulation context
Contemporary human activities increasingly compromise the biological diversity of our planet. As a consequence of habitat deterioration and fragmentation, many species have been reduced to small and often isolated populations that are at risk of extinction due to environ¬mental, demographic or genetic stochasticity. Such small-sized, partially isolated demes suffering from temporary extinctions but connected to the collective through migration are commonly known as metapopulations. In conservation biology, metapopulation theory is increasingly applied to analyse the demography and dynamics of natural populations and to estimate crucial population parameters that are subsequently used to develop measures to manage endangered species. However, most models concerning the dynamics of genetic variation and evolution of metapopulations are based on simplifying and often unrealistic assumptions. The validity of these models has rarely been evaluated, neither under experimental nor under natural conditions.
In this project, we used laboratory Drosophila melanogaster metapopulations and individual-based computer simulations to provide such validation. We studied the consequences of metapopulation structure for the dynamics of genetic diversity and differentiation, with emphasis on genetic processes such as genetic erosion and local adaptation.
Random genetic drift. In a first set of experiments, we investigated the loss of genetic variation due to genetic drift in small, isolated populations. We inferred the effective population size, the fixation rate, the heterozygosity within demes, and fixation indices within and among metapopulations. The results led to four main conclusions. First, even under standardized conditions the replicates diverged substantially. As a consequence, a sample of ten demes did not provide a representative picture of the entire metapopulation consisting of 50 demes. Second, effective population size Ne was only about half the census size. Even in a standardized laboratory environ¬ment the loss of genetic variation is strongly affected by the mating system and variance in female reproductive success. Third, the phenotypic marker chosen for its presumed neutrality was actually subject to selection. Moreover, the strength of selection depended on experimental details. Fourth, many commonly used “snapshot” estimators of effective population size substantially overestimated Ne , resulting in biased conclusions concerning the state of the metapopulation.
Migration. In a second experiment, we studied the effect of migration on genetic erosion in a metapopulation. We investigated various migrational setups, we assessed the levels of genetic diversity and genetic differentiation (fig. 3), and we inferred the effective number of migrants. Throughout, we compared the experimental results with the predictions of general metapopulation models and individual-based simulations tailored to our experimental setup. We arrived at three main conclusions. First, despite the high level of standardization, replicate metapopulations differed considerably from each other, leading to large variation in the estimates of migration rates for the same migration scenario. Second, when individuals mated before migration, the level of gene flow differed substantially between female (fig. 3A) and male (fig. 3B) migration. In other words, the effective number of migrants is strongly affected by a sex bias of migration. When compared to the island model of migration, the effective number of migrants was reduced by 50% in our unidirectional stepping-stone setup. Third, even though gene flow was sufficiently large to mitigate genetic differentiation, mean fitness declined substantially in the course of the experiment. Our findings illustrate that inferences of demographic parameters from genetic data can be unreliable, and that the evolution of a metapopulation can be strongly affected by migrant sex, the timing of migration, and the spatial configuration of subpopulations.
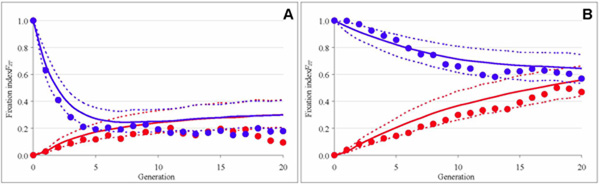
Local extinction and recolonization. In a third experiment we included both gene flow through migration and population turnover through local extinction and recolonization. As expected, population turnover increased stochasticity at the metapopulation level, resulting in a more rapid loss of diversity and higher levels of differentiation (fig. 4B) than without local extinction (fig. 4A). Therefore, effective metapopulation sizes decreased much more rapidly in the presence than in the absence of population turnover. Despite striving for constant environmental conditions, migration and colonization rates fluctuated considerably between generations, resulting in high to very high variation among replicates when population turnover occurred regularly. Compared to the effects of population turnover, the configuration of migration (stepping-stone versus migration pool) had only a relatively small effect on the outcome. Population fitness and stress tolerance both showed substantial interdemic variation, indicating that the distribution of genetic variation in a metapopulation can become very uneven when population turnover occurs regularly. Our results illustrate that relaxing experimental standardization even a little may result in large deviations from theoretical predictions, which will undoubtedly be larger yet for complex natural systems.
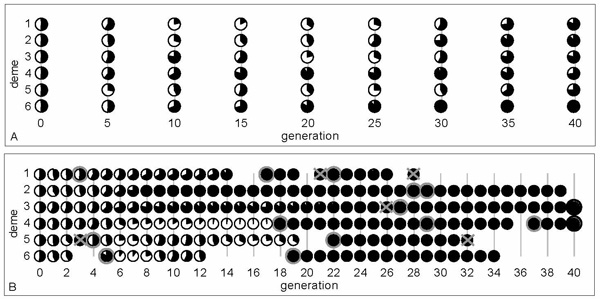
Adaptation to novel environments. The effects of environmental deterioration and habitat fragmentation may reinforce each other as habitat fragmentation causes erosion of genetic variation that may be crucial for adaptation to environmental challenges. We studied the consequences of habitat fragmentation for the adaptive potential of a metapopulation by subjecting populations with a different history of fragmentation to three novel stress environments (high temperature, ethanol, and salt). Fragmentation resulted in substantial inter-deme variation in tolerance (= viability at first exposure to stress). Contrary to expectations based on simulations, tolerance to high temperature and ethanol was higher in the fragmented than in the unfragmented populations. In line with the simulation results, the adaptive response (= change in viability after several generations of exposure to stress) was generally lower in the subdivided than in the undivided populations. The pronounced differences in tolerance and adaptive response between the three stress factors are probably related to the different genetic architectures involved in resistance to these factors (fig. 5). Our results indicate that the adaptive response of single demes may vary substantially, in particular when major genes with conditionally expressed detrimental alleles are involved (fig. 5 left).
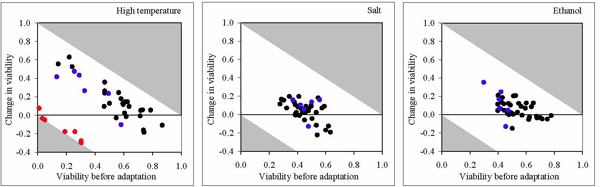
Implications for conservation biology. In all experiments we found that the average over several metapopulations matched the theoretical predictions fairly well. At the same time, the individual replicates often diverged strongly from this average. Thus, inferences based on a few subpopulations can be highly misleading. The considerable standard error of the estimates of demographic parameters encountered in all our experiments indicate that such estimates will generally have more value as a relative measure (e.g., to compare gene flow among populations) than as an absolute measure (e.g., to predict the exact numbers of migrants per generation). Perhaps surprisingly, we found no unambiguous evidence for inbreeding depression and genetic erosion due to population subdivision in our experiments. The strongest indication for genetic erosion resulted from conditionally expressed detrimental alleles. Since the occurrence of such alleles is probably an exception rather than the rule in natural populations, the consequences for the adaptive potential of natural metapopulations might be limited. Summarizing, we conclude that general theory may reasonably predict the dynamics in experimental and, perhaps, in natural populations in a qualitative way, whereas quantitative inferences are generally not possible, unless the biology and dynamics of the species under consideration are understood in considerable detail. Since such estimates may still deviate substantially from actual parameter values, prudence and wide safety margins are in order whenever they are used in practice, for example to develop a management strategy for the conservation of specific natural systems.
Last modified: | 03 December 2015 12.54 p.m. |